Contacts : Carlos Sánchez-Sánchez
Scanning electrochemical microscopy (SECM) is a technique that emerged in the late 1980s following the developments of the scanning tunneling microscopy and the atomic force microscopy. SECM is used to characterize interfacial reactivity or to perform surface modifications at the micrometer scale using an ultra-microelectrode (UME) as a local probe. This technique is now widespread and has applications in fields as diverse as biology for the characterization of living cells, molecular electrochemistry for the determination of complex reaction mechanisms or the study of fast kinetics, materials science for the development of new catalyst compositions or the study of material degradation.
Principle
SECM is based on the use of an UME that is positioned close to a substrate to obtain its topography or local reactivity from the measurement of the current flowing through the UME. By carefully selecting the polarization potential of the probe, a stationary response for the current is obtained, the value of which is controlled by the diffusion coefficient, the concentration of the electroactive species and the radius of the microelectrode. The electrochemical response of the probe will therefore make it possible to quantitatively characterize the different heterogeneities at the electrode surface. The most common operating modes are feedback modes and generator/collector modes. The spatial resolution of the SECM depends on two main parameters: the size of the probe used and the positioning distance between the probe and the substrate. It is possible to use nanoelectrodes or microelectrodes as local probes, but the main difficulty lies in controlling the distance between the probe and the substrate in order to avoid any contact between them.
Feedback modes
Feedback modes consist of using a redox mediator in solution and polarizing the microelectrode to a potential to observe the steady-state current (corresponding to the diffusion limited current). When the probe is approached from a substrate at a distance less than the size of the UME diffusion layer (i.e. a few times its radius), it is then possible to characterize the interactions between the species produced at the UME and the substrate. If the substrate is an insulator, the diffusion layer at the UME is disturbed by blocking the arrival of redox species from the bulk solution, and the current decreases as the probe-to-substrate distance decreases: this is called the negative feedback mode (Figure 1).
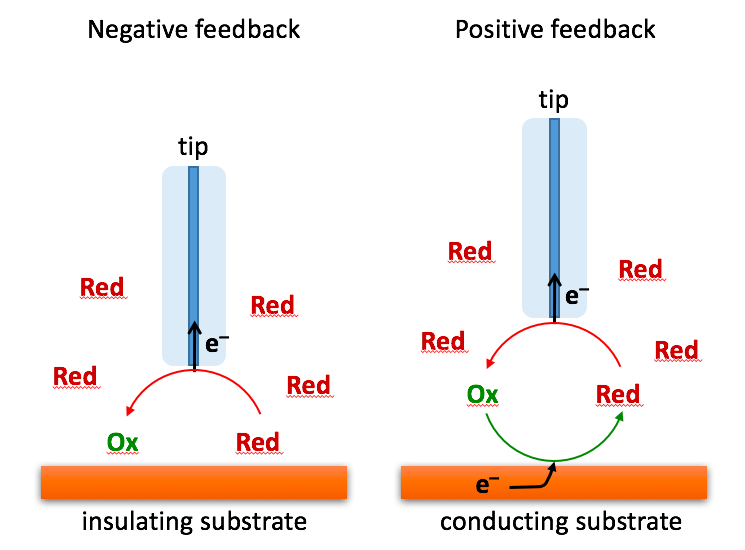
This mode makes it possible to characterize the microelectrode used as a local probe, to precisely position the probe in the vicinity of the interface to be characterized and to obtain the topography of the interface by scanning the probe above the material surface (electrochemical imaging). Conversely, if the substrate is a conductor, the species consumed at the probe can be regenerated back on the substrate and react again at the probe (Figure 1) causing a higher current than in the presence of an insulating substrate for the same distance: this is called the positive feedback mode. In addition, the higher the electrokinetic constant, the higher the current increases as the probe-o-substrate distance decreases. The approach curves thus obtained (probe current as a function of probe-to- substrate distance) allow quantitative studies of the local reactivity of the substrate. This operating mode also makes it possible to obtain maps of the local reactivity of an interface by scanning the probe in a plane parallel to the substrate as shown in Figure 2 in the case of a duplex stainless steel.1
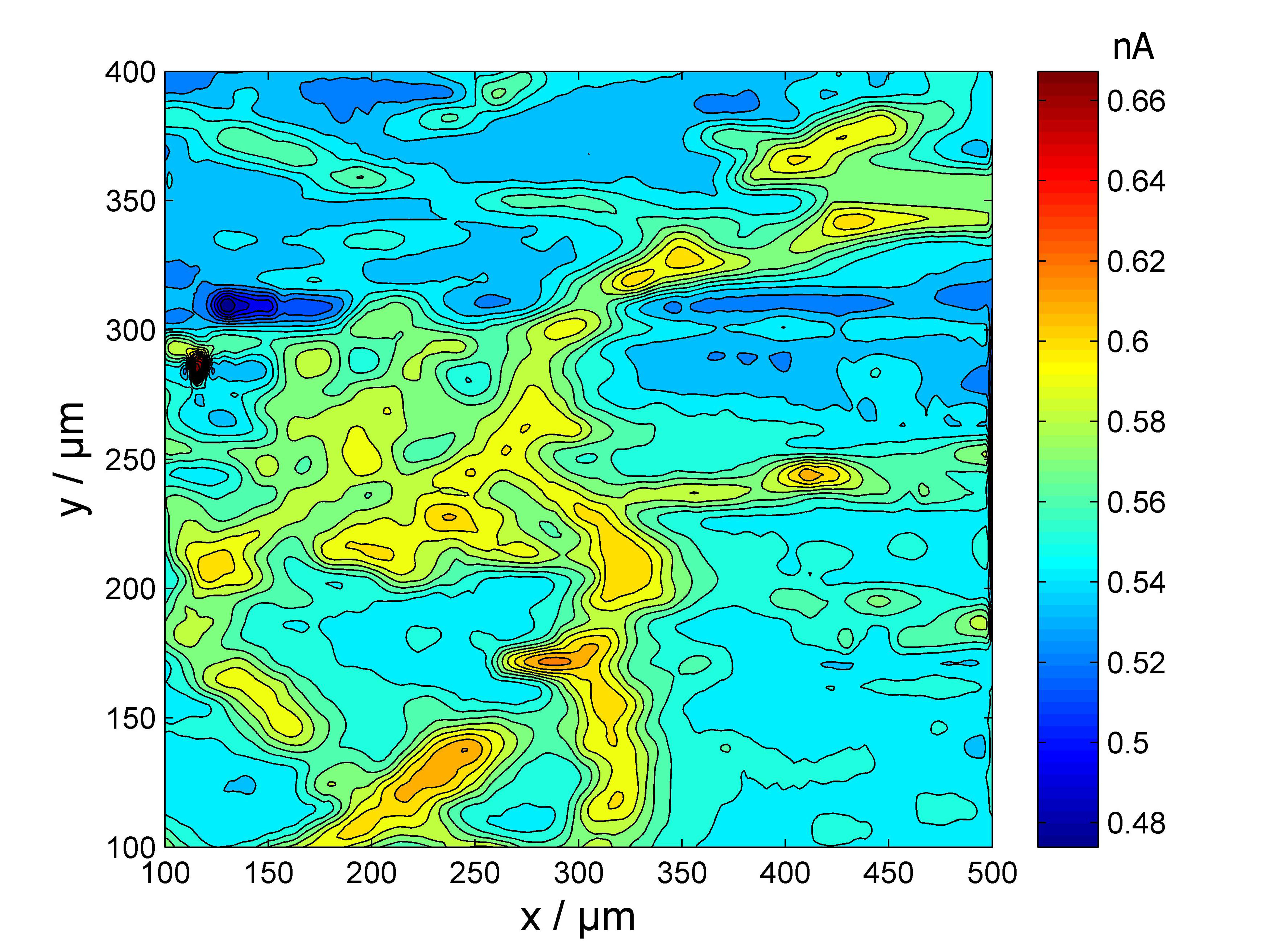
Figure 2: Local reactivity of a duplex steel imaged by SECM with ferrocene methanol as redox mediator. The most active areas correspond to the ferritic phase, the least active areas correspond to the austenitic phase.
Generator / collector modes
The second type of operation is the generator/collector mode, which consists in generating a species at one of the two electrodes (for example on the substrate) and performing detection on the other (for example on the probe). With this operating mode, it is possible to use amperometric probes (a polarized microelectrode with the appropriate potential), but also selective electrodes such as potentiometric microprobes that allow the concentration of a specific species in solution to be measured locally. This operating mode also allows the use of nanoelectrode as a local probe to characterize concentration profiles in the vicinity of larger electrodes without feedback effects.
Other Operating modes
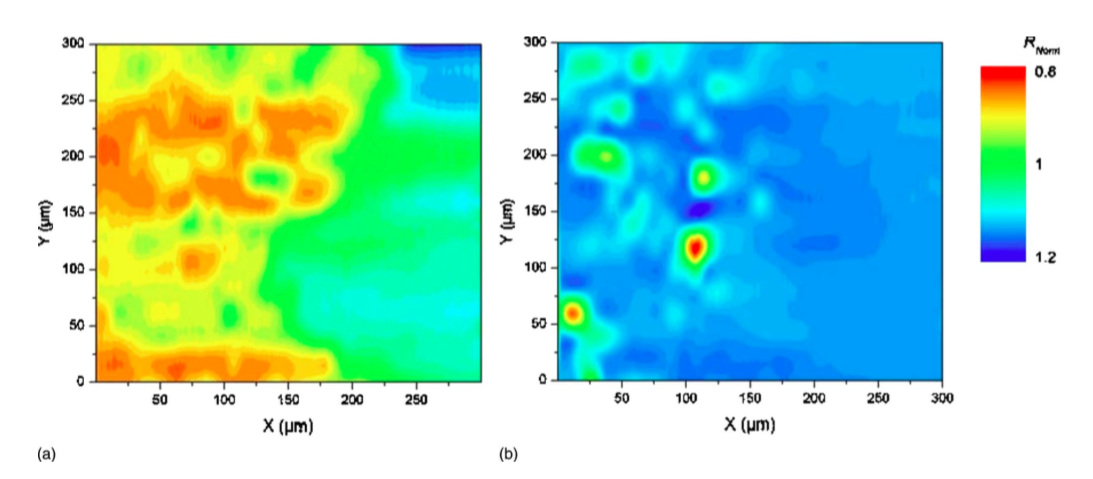
Other operating modes have been developed to overcome some experimental difficulties. When it is not possible to add a redox mediator in solution, one possible alternative is to use the electrolyte resistance measurement at the probe.2 For instance, this approach allows to characterize the formation of an oxide film on the surface of an electrode as shown in Figure 3 in the case of a magnesium electrode.3
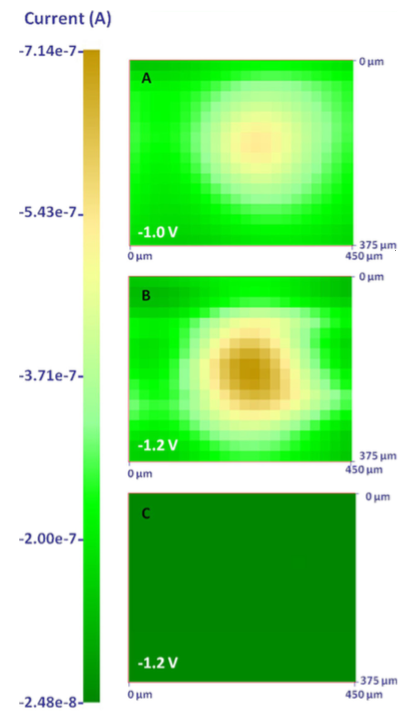
It is also sometimes easier to bring a species locally with a micro pipette so that it reacts with the substrate, for example for the characterization of catalysts.4-5 Figure 4 illustrates the use of this technique for studying the reactivity of an Ag microelectrode for the reduction of chloroform at three different potentials.
Similarly, the use of a microcapillary also allows a controlled amount of chloride ion to be supplied locally to study the rupture of the passive layer on metals and the mechanisms for the propagation of pits.6-7
Equipment available at LISE
We use commercial equipment and equipment developed in the laboratory. The latter allow us to develop the technology and adapt it to our various problems.
References
- de Assis, K. S.; de Sousa, F. V. V.; Miranda, M.; Margarit-Mattos, I. C. P.; Vivier, V.; Mattos, O. R., Assessment of electrochemical methods used on corrosion of superduplex stainless steel. Corros. Sci. 2012, 59, 71-80.
- Gabrielli, C.; Huet, F.; Keddam, M.; Rousseau, P.; Vivier, V., Scanning Electrochemical Microscopy Imaging by Means of High-Frequency Impedance Measurements in Feedback Mode. J. Phys. Chem. B 2004, 108 (31), 11620-11626.
- Baril, G.; Galicia, G.; Deslouis, C.; Pebere, N.; Tribollet, B.; Vivier, V., An impedance investigation of the mechanism of pure magnesium corrosion in sodium sulfate solutions. J. Electrochem. Soc. 2007, 154 (2), C108-C11
- Perales-Rondón, J. V.; Solla-Gullón, J.; Herrero, E.; Sánchez-Sánchez, C. M., Enhanced catalytic activity and stability for the electrooxidation of formic acid on lead modified shape controlled platinum nanoparticles. Applied Catalysis B: Environmental 2017, 201, 48-57.
- Lugaresi, O.; Perales-Rondon, J. V.; Minguzzi, A.; Solla-Gullon, J.; Rondinini, S.; Feliu, J. M.; Sanchez-Sanchez, C. M., Rapid screening of silver nanoparticles for the catalytic degradation of chlorinated pollutants in water. Appl. Catal. B-Environ. 2015, 163, 554-563.
- Aouina, N.; Balbaud-Celerier, F.; Huet, F.; Joiret, S.; Perrot, H.; Rouillard, F.; Vivier, V., A flow microdevice for studying the initiation and propagation of a single pit. Corros. Sci. 2012, 62, 1-4.
- Heurtault, S.; Robin, R.; Rouillard, F.; Vivier, V., Initiation and propagation of a single pit on stainless steel using a local probe technique. Faraday Discuss. 2015, 180, 267-282.